Home » Student Resources » Online Chemistry Textbooks » CH103: Allied Health Chemistry » CH103 – Chapter 7: Chemical Reactions in Biological Systems
MenuCH103: Allied Health Chemistry
CH103 – Chapter 7: Chemical Reactions in Biological Systems
This text is published under creative commons licensing. For referencing this work, please click here.
7.1 What is Metabolism?
7.2 Common Types of Biological Reactions
7.3 Oxidation and Reduction Reactions and the Production of ATP
7.4 Reaction Spontaneity
7.5 Enzyme-Mediated Reactions
7.6 Introduction to Pharmacology
7.7 Chapter Summary
7.8 References
7.1 What is Metabolism?
Metabolism is the set of life-sustaining chemical reactions in organisms. We have seen examples of metabolic processes in the primary and secondary metabolites covered in Chapter 6. Overall, the three main purposes of metabolism are: (1) the conversion of food to energy to run cellular processes; (2) the conversion of food/fuel to building blocks for proteins, lipids, nucleic acids, and carbohydrates; and (3) the elimination of waste products. These enzyme-catalyzed reactions allow organisms to grow and reproduce, maintain their structures, and respond to their environments. (The word metabolism can also refer to the sum of all chemical reactions that occur in living organisms, including digestion and the transport of substances into and between different cells, in which case the above described set of reactions within the cells is called intermediate metabolism.)
Metabolic reactions may be categorized as catabolic – the breaking down of compounds (for example, the breaking down of proteins into amino acids during digestion); or anabolic – the building up (synthesis) of compounds (such as proteins, carbohydrates, lipids, and nucleic acids). Usually, catabolism releases energy, and anabolism consumes energy.
Figure 7.1 Catabolic and Anabolic Reactions. Catabolic reactions involve the breakdown of molecules into smaller components, whereas anabolic reactions build larger molecules from smaller molecules. Catabolic reactions usually release energy whereas anabolic processes usually require energy.
Figure is modified from Metabolism Overview
The chemical reactions of metabolism are organized into metabolic pathways, in which one chemical is transformed through a series of steps into another chemical, each step being facilitated by a specific enzyme. Enzymes are crucial to metabolism because enzymes act as catalysts – they allow a reaction to proceed more rapidly. In addition, enzymes can provide a mechanism for cells to regulate the rate of a metabolic reaction in response to changes in the cell’s environment or to signals from other cells, through the activation or inhibition of the enzymes activity. Enzymes can also allow organisms to drive desirable reactions that require energy that will not occur by themselves, by coupling them to spontaneous reactions that release energy. Enzyme shape is critical to the function of the enzyme as it determines the specific binding of a reactant. This can occur by a lock and key model where the reactant is the exact shape of the enzyme binding site, or by an induced fit model, where the contact of the reactant with the protein causes the shape of the protein to change in order to bind to the reactant.
Figure 7.2 Mechanisms of Enzyme-Substrate Binding. (A) In the Lock and Key Model, substrates fit into the active site of the enzyme with no further modifications to the enzyme shape required. (B) In the Induced Fit Model, substrate interaction with the enzyme causes the shape of the enzyme to change to better fit the substrate and mediate the chemical reaction.
Figure 7.2A was modified from Socratic and Figure 7.2B was modified from Concepts in Biology
7.2 Common Types of Biological Reactions
Within biological systems there are six major classes of biochemical reactions that are mediated by enzymes. These include group transfer reactions, the formation/removal of carbon-carbon double bonds, isomerization reactions, ligation reactions, hydrolysis reactions, and oxidation-reduction reactions. This section will give you a brief introduction to these six types of reactions and then the following section will focus more in-depth on oxidation-reductions and how they are critical for the formation of the major form of cellular energy, adenosine triphosphate (ATP). Note that all of these reaction types require an enzyme catalyst (usually a specific protein) to speed up the rate of the reactions within biological systems.
Group Transfer Reactions
In group transfer reactions, a functional group will be transferred from one molecule that serves as the donor molecule to another molecule that will be the acceptor molecule. The transfer of an amine functional group from one molecule to another is common example of this type of reaction and is shown in Figure 7.3 below.
Figure 7.3 Transfer of an Amine Functional Group. A common group transfer reaction in biological systems is one that is used to produce α-amino acids that can then be used for protein synthesis. In this reaction, one α-amino acid serves as the donor molecule and an α-keto acid (these molecules contain a carboxylic acid functional group and a ketone functional group separated by one α-carbon) serves as the acceptor. In the acceptor molecule, the carbonyl oxygen is replaced with the amine functional group, whereas in the donor molecule, the amine functional group is replaced by an oxygen forming a new ketone functional group.
The Formation/Removal of Carbon-Carbon Double Bonds
Reactions that mediate the formation and removal of carbon-carbon double bonds are also common in biological systems and are catalyzed by a class of enzymes called lyases. The formation or removal of carbon-carbon double bonds is also used in synthetic organic chemistry reactions to create desired organic molecules. One of these types of reactions is called a hydrogenation reaction, where a molecule of hydrogen (H2) is added across a C-C double bond, reducing it to a C-C single bond. If this is done using unsaturated oils, the unsaturated fats can be converted into saturated fats (Figure 7.4). This type of reaction is commonly done to produce partially hydrogenated oils converting them from liquids at room temperature into solids. Margarines made from vegetable oil are made in this manner. Unfortunately, a by-product of this reaction can be the formation of TAGS containing trans double bonds. Once the health hazards of consuming trans fats was recognized, the Food and Drug Administration (FDA) placed a ban on the inclusion of trans fats in food products. This ban was enacted in the summer of 2015 and gave food-makers three years to eliminate them from the food supply, with a deadline of June 18, 2018.
Figure 7.4 Hydrogenation of Oils to Produce Margarine. Unsaturated oils can by partially or fully hydrogenated to produce the saturated fatty acids to produce margarines that will remain solid at room temperature. The addition of the new hydrogen atoms to create the saturated hydrocarbons are shown in yellow in the final product.
Upper photo provided by Cottonseed Oil and lower photo provided by Littlegun
Isomerization Reactions
In isomerization reactions a single molecule is rearranged such that it retains the same molecular formula but now has a different bonding order of the atoms forming a structural or stereoisomer. The conversion of glucose 6-phosphate to fructose 6-phosphate is a good example of an isomerization reaction and is shown in figure 7.5
Figure 7.5 Isomerization of Glucose 6-phosphate to Fructose 6-phosphate.
Ligation Reactions
Ligation reactions use the energy of ATP to join two molecules together. An example of this kind of reaction is the joining of the amino acid with the transfer RNA (tRNA) molecule during protein synthesis. During protein synthesis the tRNA molecules bring each of the amino acids to the ribosome where they can be incorporated into the newly growing protein sequence. To do this, the tRNA molecules must first be attached to the appropriate amino acid. Specific enzymes are available called amino acyl – tRNA synthetases that mediate this reaction. The synthetase enzymes use the energy of ATP to covalently attach the amino acid to the tRNA molecule. A diagram of this process is shown in Figure 7.6. For each of the 20 amino acids, there is a specific tRNA molecule and a specific synthetase enzyme that will ensure the correct attachment of the correct amino acid with its tRNA molecule.
Figure 7.6 Ligation Reaction Covalently Attaching Methionine with the Appropriate tRNA. The amino-acyl tRNA synthetase enzyme for methionine (shown in blue) covalently attaches methionine (light pink) with the methionine tRNA molecule (dark pink). This reaction requires the energy provided from the breakdown of the ATP molecule into AMP, releasing energy with the breakdown of the phosphate bonds into two inorganic phosphate ions (2 Pi).
Figure provided by the Kahn Academy
Hydrolysis Reactions
The classification of hydrolysis reactions include both the forward reactions that involve the addition of water to a molecule to break it apart or the reverse reaction involving the removal of water to join molecules together, termed dehydration synthesis (or condensation) (Figure 7.7). When water is added to a molecule to break it apart into two molecules this reaction is called hydrolysis. The term ‘lysis‘ means to break apart, and the term ‘hydro‘ refers to water. Thus, the term hydrolysis means to break apart with water. The reverse of that reaction involves the removal of water from two molecules to join them together into a larger molecule. Since the two molecules are losing water, they are being dehydrated. Thus, the formation of molecules through the removal of water is known as dehydration synthesis. Since water is also a by-product of these reactions, they are also commonly referred to as condensation reactions. As we have seen in Chapter 6, the formation of the major classes of macromolecules in the body (proteins, carbohydrates, lipids, and nucleic acids) are formed through dehydration synthesis where water is removed from the molecules (Figure 7.x). During normal digestion of our food molecules, the major macromolecules are broken down into their building blocks through the process of hydrolysis.
Figure 7.7 Hydrolysis and Dehydration Synthesis. The reactions of hydrolysis mediate the breakdown of larger polymers into their monomeric building blocks by the addition of water to the molecules. The reverse of the reaction is dehydration synthesis, where water is removed from the monomer building blocks to create the larger polymer structure.
As you learned in Chapter 6, the major macromolecules are built by putting together repeating monomer subunits through the process of dehydration synthesis. Interestingly, the organic functional units used in the dehydration synthesis processes for each of the major types of macromolecules have similarities with one another. Thus, it is useful to look at the reactions together (Figure 7.8)
Figure 7.8 Dehydration Synthesis Reactions Involved in Macromolecule Formation. The major organic reactions required for the biosynthesis of lipids, nucleic acids (DNA/RNA), proteins, and carbohydrates are shown. Note that in all of the reactions, there is a functional group that contains two electron withdrawing groups (the carboxylic acid, phosphoric acid and the hemiacetal each have two oxygen atoms attached to a central carbon or phosphorus atom). This forms a reactive partially positive center atom (carbon in the case of the carboxylic acid and hemiacetal, or phosphorus in the case of the phosphoric acid) that can be attacked by the electronegative oxygen or nitrogen from an alcohol or amine functional group.
The formation of esters and the related compounds, amides, phosphoesters, and acetals are formed by dehydration synthesis, involving the loss of water. The reaction mechanisms for each of these reactions is very similar. Let’s take a look at the formation of the ester linkage as an example (Figure 7.9).
Figure 7.9 Reaction Mechanism of Ester Formation. (1) This reaction mechanism is set up by the nature of carboxylic acid functional group. The presence of the carbonyl oxygen and the alcohol functional groups create an electron withdrawing situation, where the electronegative oxygen atoms pull the electrons away from the central carbon atom. This creates a very polar situation, where the central carbon has a strong partial positive character. (2) The strong partial positve character of the central carbon atom of the carboxylic acid attracts one of the lone pair electron groups from the alcohol functional group, shown in red. This enables a new covalent bond to form between the alcohol functional group and the carboxylic acid functional group. This creates an intermediate that has five bonds attached to the central carbon and three bonds attached to the oxygen atom of the incoming alcohol. (3) The intermediate with five bonds to the central carbon is unstable and wouldn’t normally form, however the presence of the carbonyl oxygen makes the reaction more favorable. It will be able to temporarily absorb the extra electron potential around the central carbon atom, due to its electronegative character and the double bond will temporarily shift up onto the central oxygen forming a lone pair intermediate. (4) The extra lone pair on the carbonyl oxygen shifts back down to reform the double bond with the central carbon. (5) This causes the shared electron pair between the central carbon atom and the original alcohol functional group to shift over to the alcohol, breaking the covalent bond. (6) The extra lone pair of electrons on the free alcohol group take the proton from the new incoming alcohol group forming a molecule of water and the final ester structure.
All of the dehydration synthesis reactions shown for the major macromolecules have a similar reaction mechanism to that shown for the ester bond formation. Notice that the reverse of the reactions show mediate the hydrolysis of the bond linkage by the addition of the water molecule across the bond. This restores the original functional groups, a carboxylic acid and an alcohol in the case of the ester.
Oxidation-Reduction Reactions
An oxidation-reduction (redox) reaction is a type of chemical reaction that involves a transfer of electrons between two atoms or compounds. The substance that loses the electrons is said to be oxidized, while the substance that gains the electrons is said to be reduced. Redox reactions always have to occur together. If one molecule is oxidized, then another molecule has to be reduced (ie. electrons don’t appear out of thin air to be added to a compound, they always have to come from somewhere!).
The change in electron composition can be evaluated in the change of the oxidation state (or number) of an atom. Therefore, an oxidation-reduction reaction is any chemical reaction in which the oxidation state (number) of a molecule, atom, or ion changes by gaining or losing an electron. We will learn how to evaluate the oxidation state of a molecule within this section. Overall, redox reactions are common and vital to some of the basic functions of life, including photosynthesis, respiration, combustion, and corrosion or rusting.
As shown in Figure 7.10, an easy mnemonic for helping you remember which member gains electrons and which member loses electrons is ‘LEO the lion says GER’, where LEO stands for Lose Electrons = Oxidized and GER stands for Gain Electrons = Reduced.
Figure 7.10. The Rules of Oxidation and Reduction. The mnemonic LEO the lion says GER is a helpful way to remember the major concepts of Oxidation-Reduction reactions, noting that when a molecule Loses Elections it is Oxidized (LEO), and when a molecule Gains Electrons it is Reduced (GER).
Rules for Assigning Oxidation States
The oxidation state of an element corresponds to the number of electrons, e–, that an atom loses or gains during an ionic bond, or appears to lose/grain when joining in covalent bonds with other atoms in compounds. In determining the oxidation state of an atom, there are seven guidelines to follow:
- The oxidation state of an atom in its elemental form is 0. (This includes elemental forms that occur as diatomic molecules. For example, each oxygen in a molecule of O2, has an oxidation state = 0.)
- The total oxidation state of all atoms in a neutral species is 0 and in an ion is equal to the ion charge. (For example, the overall oxidation state of NaCl = 0, even though the oxidation state of Na+ in this bond is +1 and the oxidation state of the chlorine atom, Cl–, is -1. When you add them together they equal 0. In the case of an ion, the overall charge is always indicated. For example, the overall charge of a OH– ion is -1, while the oxygen in the OH– has a -2 oxidation state and the hydrogen has a +1 oxidation state.)
- Group 1 metals have an oxidation state of +1 and Group 2 an oxidation state of +2 when they are involved in ionic bonding.
- The oxidation state of fluorine is -1 in compounds
- Hydrogen generally has an oxidation state of +1 in compounds
- Oxygen generally has an oxidation state of -2 in compounds
- In binary metal compounds, Group 17 (or 7A) elements have an oxidation state of -1, Group 16 (or 6A) of -2, and Group 15 (or 5A) of -3.
- The oxidation states of other atoms are calculated based on rules 1-7.
Combustion reactions almost always involve oxygen in the form of O2, and are almost always exothermic, meaning they produce heat. Chemical reactions that give off light and heat are colloquially referred to as “burning.” Complete combustion of carbon compounds results in the production of carbon dioxide (CO2) and water (H2O). Note that carbon can exist in a range of oxidation states, typically from -4 to +4. The burning of fuels that provides the energy to maintain our civilization and the metabolism of foods that furnish the energy that keeps us alive both involve redox reactions.
All combustion reactions are also redox reactions. The general formula for a combustion reaction is:
CxHy + O2 → CO2 + H2O
One specific example is the burning of acetylene (C2H2) in torches:
2C2H2 + 5O2 → 4CO2 + 2H2O
Oxygen (in its elemental form) is a crucial reactant in combustion reactions, and it is also present in the products. Combustion reactions can be evaluated for their redox potential by assigning oxidation numbers to each element in the reaction:
Overall in combustion reactions, the hydocarbon (in this case, acetylene) is oxidized by molecular oxygen to produce carbon dioxide and water. In the process, oxygen is reduced.
In respiration, the biochemical process by which the oxygen we inhale in air oxidizes foodstuffs to carbon dioxide and water, redox reactions provide energy to living cells. A typical respiratory reaction is the oxidation of glucose (C6H12O6), a simple sugar.
C6H12O6 + 6O2 → 6CO2 + 6H2O
Inside the body, the reaction is controlled to harvest the energy released so that it can be utilized for the production of ATP. Some of the energy released is also used to generate heat for the organism, but not in the rapid form of a combustion reaction that produces fire. Note that in redox reactions involving hydrocarbons, that they hydrogens are usually removed with the electrons. Thus, they can be used as an indicator for which molecules are being oxidized and which are being reduced. For example, in the reaction above the glucose molecule (C6H12O6) is losing the hydrogens as it is converted to carbon dioxide. As the glucose losing the hydrogens, it is also losing electrons and thus, the glucose is the oxidized component. Similarly, the oxygen on the reactant side is being converted into the water on the product side where it is gaining the hydrogens, and also gaining electrons. Thus, in this reaction, the oxygen is being reduced to water.
Note that reaction classifications can also overlap and fall into more than one category. For example, the hydrogenation reaction presented above in the removal of carbon-carbon double bonds is also an example of a redox reaction. In that reaction, the hydrocarbons are gaining hydrogens and also electrons, as the double bonds are removed forming the saturated hydrocarbons. Thus, the TAGs are being reduced in this reaction. In this case, the oxidized component (H2) is being entirely used up in this process.
7.3 Oxidation and Reduction Reactions and the Production of ATP
As seen above, organic molecules that contain a lot of carbon and hydrogen bonds have high energy potential and ability to be oxidized to CO2 and water. Of all the major macromolecules, fats have the highest hydrocarbon content and thus, contain the largest energy potential (9 Cal/g). Proteins and carbohydrates have many more heteroatoms, such as oxygen and nitrogen incorporated into their structures and have less energy potential (4 Cal/g for both proteins and carbohydrates). In this section, we will cover the oxidation potential of organic molecules with oxygen-containing functional groups.
Alcohol Functional Groups
Alcohol functional groups contain the greatest oxidative potential of all the oxygen containing functional groups. The reactivity of alcohols depend on the number of carbon atoms attached to the specific carbon atom that is attached to the -OH group. Alcohols can be grouped into three classes on this basis.
- A primary (1°) alcohol is one in which the carbon atom (in red) with the OH group is attached to one other carbon atom (in blue). Its general formula is RCH2OH.

- A secondary (2°) alcohol is one in which the carbon atom (in red) with the OH group is attached to two other carbon atoms (in blue). Its general formula is R2CHOH.

- A tertiary (3°) alcohol is one in which the carbon atom (in red) with the OH group is attached to three other carbon atoms (in blue). Its general formula is R3COH.

Some alcohols can undergo oxidation reactions. Remember in redox reactions, the component of the reaction that is being oxidized is losing electrons (LEO) while the molecule receiving the electrons is being reduced (GER). In organic reactions, the flow of the electrons usually follows the flow of the hydrogen atoms. Thus, the molecule losing hydrogens is typically also losing electrons and is the oxidized component. The molecule gaining electrons is being reduced. For alcohols, both primary and secondary alcohols can be oxidized. Tertiary alcohols, on the other hand, cannot be oxidized. In many oxidation reactions the oxidizing agent is shown above the reaction arrow as [O]. The oxidizing agent can be a metal or another organic molecule. In the reaction, the oxidizing agent is the molecule that is reduced or accepts the electrons.
In alcohol oxidation reactions, the hydrogen from the alcohol and a hydrogen that is attached to the carbon that has the alcohol attached, along with their electrons, are removed from the molecule by the oxidizing agent. Removal of the hydrogens and their electrons results in the formation of a carbonyl functional group. In the case of a primary alcohol, the result is the formation of an aldehyde. In the case of a secondary alcohol, the result is the formation of a ketone. Note that for a tertiary alcohol, that the carbon attached to the alcohol functional group does not have a hydrogen atom attached to it. Thus, it cannot undergo oxidation. When a tertiary alcohol is exposed to an oxidizing agent, no reaction will occur.
Notice that for the primary alcohol that undergoes oxidation, that it still retains a hydrogen atom that is attached to the carbonyl carbon in the newly formed aldehyde. This molecule can undergo a secondary oxidation reaction with an oxidizing agent and water, to add another oxygen atom and remove the carbonyl hydrogen atom. This results in the formation of a carboxylic acid.
Example Problem:
Write an equation for the oxidation of each alcohol. Use [O] above the arrow to indicate an oxidizing agent. If no reaction occurs, write “no reaction” after the arrow.
- CH3CH2CH2CH2CH2OH
-
-
Solution
The first step is to recognize the class of each alcohol as primary, secondary, or tertiary.
-
This alcohol has the OH group on a carbon atom that is attached to only one other carbon atom, so it is a primary alcohol. Oxidation forms first an aldehyde and further oxidation forms a carboxylic acid.
-
This alcohol has the OH group on a carbon atom that is attached to three other carbon atoms, so it is a tertiary alcohol. No reaction occurs.
-
This alcohol has the OH group on a carbon atom that is attached to two other carbon atoms, so it is a secondary alcohol; oxidation gives a ketone.
More Practice:
Write an equation for the oxidation of each alcohol. Use [O] above the arrow to indicate an oxidizing agent. If no reaction occurs, write “no reaction” after the arrow.
Aldehyde and Ketone Functional Groups
As shown above in the alcohol section, aldehydes can undergo oxidation to produce a coarboxylic acid. This is because the carbonyl carbon atom still retains a hydrogen atom that can be removed and replaced with an oxygen atom. Ketones on the other hand, do not contain a hydrogen atom bound to the carbonyl carbon atom. Thus, they cannot undergo further oxidation. As noted above, ketones that are exposed to an oxidizing agent will have no reaction.
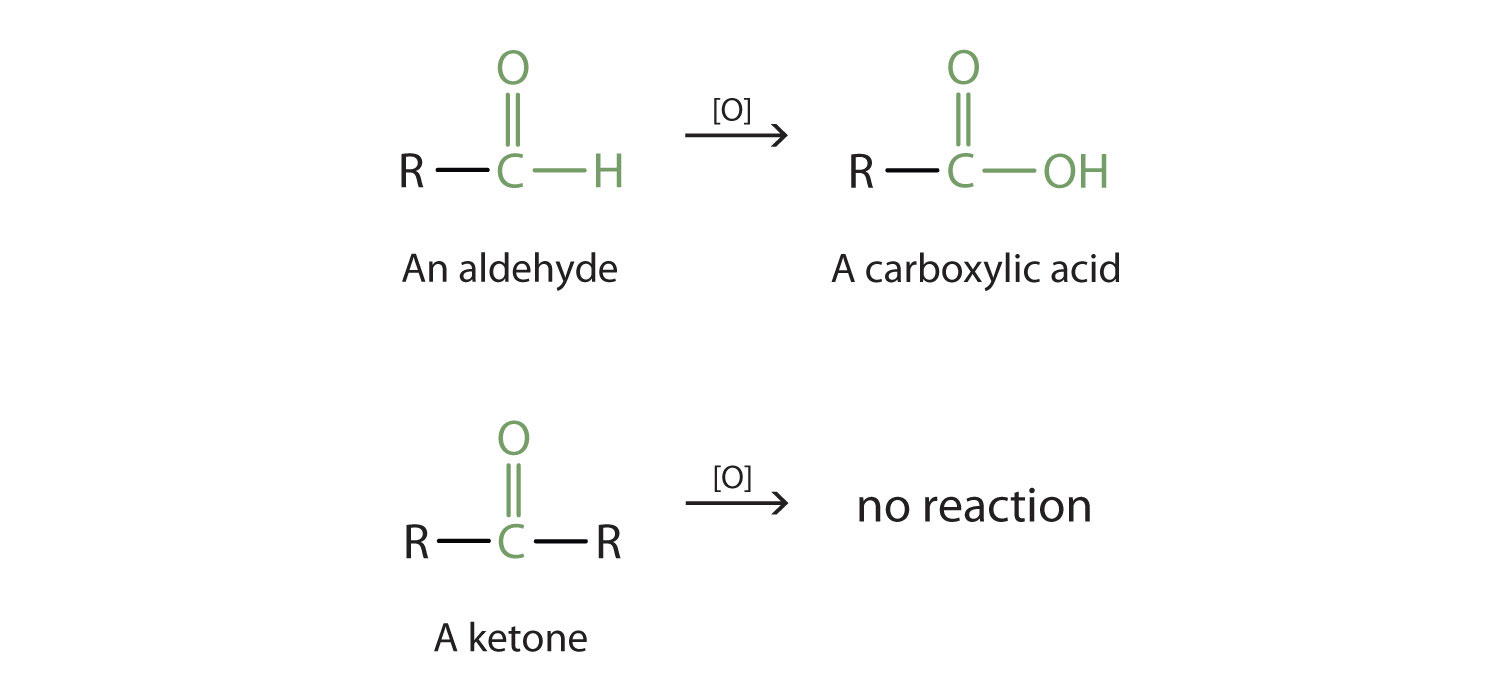
Reduction Reactions
Reduction reactions with aldehydes and ketones revert these compounds to primary alcohols in the case of aldehydes and secondary alcohols in the case of ketones. They are essentially the reverse reactions of the alcohol oxidation reactions.
For example, with the aldehyde, ethanal you get primary alcohol, ethanol:
Notice that this is a simplified equation where [H] means “hydrogen from a reducing agent”. In general terms, reduction of an aldehyde leads to a primary alcohol.
Reduction of a ketone, such as propanone will give you a secondary alcohol, such as 2-propanol:
Reduction of a ketone leads to a secondary alcohol.
To Your Health: The Physiological Effects of Alcohols
Methanol is quite poisonous to humans. Ingestion of as little as 15 mL of methanol can cause blindness, and 30 mL (1 oz) can cause death. However, the usual fatal dose is 100 to 150 mL. The main reason for methanol’s toxicity is that we have liver enzymes that catalyze its oxidation to formaldehyde, the simplest member of the aldehyde family:

Formaldehyde reacts rapidly with the components of cells, coagulating proteins in much the same way that cooking coagulates an egg. This property of formaldehyde accounts for much of the toxicity of methanol.
Organic and biochemical equations are frequently written showing only the organic reactants and products. In this way, we focus attention on the organic starting material and product, rather than on balancing complicated equations.
Ethanol is oxidized in the liver to acetaldehyde:

The acetaldehyde is in turn oxidized to acetic acid (HC2H3O2), a normal constituent of cells, which is then oxidized to carbon dioxide and water. However, it should be noted that acetaldehyde is toxic and can build up to dangerous levels with chronic, high use of alcohol. The liver is the major site for alcohol metabolism, thus, chronic drinking can lead to liver cirrhosis, a dangerous disease that converts normal liver tissue into scar tissue that can no longer function.
Ethanol itself can also be toxic to humans. The rapid ingestion of 1 pt (about 500 mL) of pure ethanol would kill most people, and acute ethanol poisoning kills several hundred people each year—often those engaged in some sort of drinking contest. Ethanol freely crosses into the brain, where it depresses the respiratory control center, resulting in failure of the respiratory muscles in the lungs and hence suffocation. Ethanol is believed to act on nerve cell membranes, causing a diminution in speech, thought, cognition, and judgment.
While heavy use of alcohol increases the risk of liver disease and for some forms of cancer, some reports that suggest that moderate use of alcohol (no more than 1 drink/day for women of any age and men aged 65 or older; no more than 2 drinks for men under 65) can have some benefits in reducing the risk of heart disease and ischemic stroke. For more information, the Mayo Clinic has a detailed website that outlines the risks and potential benefits of alcohol consumption.
Rubbing alcohol is usually a 70% aqueous solution of isopropyl alcohol. It has a high vapor pressure, and its rapid evaporation from the skin produces a cooling effect. It is toxic when ingested but, compared to methanol, is less readily absorbed through the skin and can thus be used topically for muscle soreness.
Overall, the processes of oxidation and reduction are critical for life. This is because the oxidation of our food molecules provides enough energy for the cells in our body to recycle the major source of energy for cellular metabolism and other energetic reactions required for life, adenosine triphosphate (ATP) (Figure 7.11). This is also a major building block for RNA biosynthesis. When the phosphate bonds are hydrolyzed to produce ADP and water, there is a large release of energy. The ability of the ATP phosphoester bonds to undergo hydrolysis is the reason it is such a great energy source! The hydrolysis of the last phosphate bond releases the most energy and is most commonly coupled with other reactions that require energy (as seen with the Na+/K+ ATPase Pump processes during the generation of the negative resting potential in neurons in Chapter 3). Once the ATP molecule is broken down to adenosine diphosphate (ADP), it needs to be recycled back to the ATP molecule. The average human will typically use their body weight in ATP every day (60-75 kg)!! However, the amount of ATP/ADP within an average human is only approximately 0.10 mol. This means that each ATP molecule must be recycled between 500-750 times each day! This requires a large energy input that comes from the electrons (e-) and protons (H+) harvested during the oxidation of our food molecules (Figure 7.11).
Figure 7.11 ATP/ADP Recycling. ATP is the main energy source within the body. Cleavage of the high energy phosphate bonds releases large amounts of energy that are used for neuron function, muscle contraction, and other metabolic processes in the body. In fact, so much energy is needed to run the human body that each ATP molecule must be recycled an average of 500-750 times per day.
Thought question 1: If there is 0.1 mol of ATP in the body at any one time, how many molecules of ATP are present in the human body? If each of these molecules is recycled 750 times (on a particularly active day), how many chemical reactions take place just to regenerate this energy source?
Thought question 2: If there is 0.1 mol of ATP in the body at any one time and the molar mass of ATP 507.181 g/mol, what is the mass in grams of ATP present in the body?
The bulk of the ATP molecules are recycled inside the mitochondria. Mitochondria are small organelles within the cell that are thought to have originated as a bacterial symbiont within the cell (Figure 7.12). Mitochondria have a double membrane, with the innermembrane being highly convoluted and folded, providing a lot of surface area for embedded membrane proteins. Mitochondria also contain their own circular DNA which is reminiscent of their bacterial origin.
Figure 7.12 Basic Structure of a Mitochondria. The mitochondria are commonly known as the powerhouse of the cell, as this is the primary site where ADP is recycled into ATP.
Figure by: National Human Genome Research Institute
As food is ingested, the large macromolecules (proteins, carbohydrates, and lipids) are digested into their monomer units. The monomer units, such as glucose from starch or fatty acids from TAGs, are delivered to cells where they can be used as an energy source to regenerated ATP from ADP. This regeneration process is called oxidative phosphorylation. The term oxidative is used because the food molecules are fully oxidized to carbon dioxide (CO2) during the process to release energy. Phosphorylation is the process of adding a phosphate group to a molecule. In this case, the energy that is harvested from the oxidation of the food molecules, specifically the electrons and protons, is being used to phosphorylate ADP back into an ATP (Figure 7.13).
Most of the oxidation reactions in the breakdown of food molecules take place in the interior of the mitochondria, called the matrix. The electrons (e-) and protons (H+) harvested in this process are transported by carrier molecules to the innermembrane of the mitochondria (Figure 7.13). Once they reach the innermembrane, the electrons are delivered to a series of proton pump proteins. Using the energy of the electrons, the proton pumps move H+ against their concentration gradient into the intermembrane space of the mitochondria. The intermembrane space is the area beetween the two membranes of the mitochondria (the innermembrane and the outermembrane).
As the intermembrane space becomes full of protons, this creates a gradient potential. You can think of a gradient potential in a similar way that humans will use the power of water in a dam to generate electricity. The dammed water holds potential energy when there is high water in the dam. When the dam is opened in a controlled way to allow water to flow out, the power of the dammed water moving from an area of high concentration to an area of low concentration is used to turn turbines that can generate electricity. Similarly, in the mitochondria, the protons that are concentrated in the intermembrane space also have potential energy. Energy from this proton gradient is used to produce ATP through a proton channel protein called the ATP synthase. When the ATP synthase is bound to ADP and a phosphate ion (PO43-), the channel opens allowing the flow of H+ ions to move through the channel. The movement of the H+ ions through the protein causes the protein to turn like a cog wheel or a turbine. This turning process enables ADP and PO43- to be joined together forming ATP.
The electrons that have been used to generate the proton gradient end up reducing molecular oxygen (O2) into water (H2O). The oxygen supplied for this process is the oxygen that we breath in through our lungs. Thus, the oxidative phosphorylation process is also known as cellular respiration. This is, in fact, why breathing is crucial for our survival. Without a steady supply of oxygen to accept the electrons moving through the electron transport chain of proton pumps, the electrons would back up and get stuck inside the proton pumps like a traffic jam, blocking the further movement of protons into the intermembrane space. Without the proton gradient, the production of ATP would no longer be possible. The first organ to run out of ATP during a lack of oxygen is the brain. If you block the passage of oxygen-carrying blood to the brain, a person will pass out in as little as 5 – 10 seconds!
A summary of the oxidative phosphorylation process is shown in Figure 7.13.
Figure 7.13 Oxidative Phosphorylation in the Mitochondria. (1) Electrons from food molecules are carried to protein pumps in the innermembrane of the mitochondria. (2) Using the energy of the electrons, the protein pumps move protons (H+) into the intermembrane space, where the protons become concentrated. (Note that the protons also came from the food molecules during the oxidation process – hydrogens and electrons often move together during oxidation!). (3) The electrons are passed through all of the pumps until most of the energy housed in them is spent. Then they are used in combination with protons, to reduce oxygen (O2) into water (H2O). (4) The H+ gradient carries energy potential, just like water that has been dammed. When it flows through the ATP synthase protein, the protein turns like a cog wheel and is able to regenerate ATP.
This Figure was adapted from: Geraldine Adele Lewis
7.4 Reaction Spontaneity
In the previous sections, you have learned an important key takeaway about chemical reactions: In all types of chemical reactions, bonds are broken and reassembled into new products. You have also learned that energy is stored in chemical bonds. This is the energy of attraction between the atoms involved in the chemical bond and is called the bond energy. Thus, to break a chemical bond requires energy (ie the attraction of the atoms for each other has to be overcome in order to pull them apart). Similarly, when new bonds are formed, energy is released as the formation of the bond creates a more stable situation for each of the atoms involved in the bonds. Overall,
Breaking Bonds = Requires Energy
Forming Bonds = Releases Energy
It is also of note, that not all chemical bonds are created equally. We have learned in previous chapters that some atoms tend to form ionic bonds where they will fully donate or accept electrons between the atoms involved in the bond. Others form covalent bonds where they share electrons between the atoms and sometimes this sharing is unequal creating a polar covalent bond. Thus, for each type of chemical bond, the bond energy will be different. Each molecule will have its own characteristic bond energies. Other factors that influence the overall energy required for reactions are the physical state of the reactants and products (ie solid, liquid, or gaseous), the temperature of the reaction, and the amounts of reactants and products present. Thus, when assessing whether or not a reaction will proceed spontaneously, it is necessary to determine which side of the equation energy will either be required or released. If the reaction requires more energy to break the bonds on the reactant side than is formed on the product side the reaction is said to be endergonic and will require an input of energy. This type of reaction will not be spontaneous. If the reaction produced by the formation of new bonds on the product side is more that the energy required to break the chemical bonds on the reactant side of the equation, the reaction will release energy and is said to be exergonic. Exergonic reactions will happen spontaneously. Reaction spontaneity can be evaluated graphically for a chemical reaction using a Gibb’s Free Energy diagram (Figure 7.14). By measuring Changes in the Gibbs Free Energy (ΔG) between the products and the reactants of a reaction it is possible to determine the amount of free energy available to do useful work. If the ΔG is negative the reaction is spontaneous, and if the ΔG is positive the reaction is not spontaneous. If ΔG = 0 the reaction is in a state of equilibrium, meaning that the forward reaction is happening at an equal rate as the reverse reaction and there is no net gain or loss of reactants and products.
Note that reaction spontaneity does not depend on the presence or absence of an enzyme (ie. the presence of an enzyme cannot change a nonspontaneous reaction into a spontaneous reaction.)
Figure provided by The Khan Academy
7.5 Enzyme-Mediated Reactions
The single most important property of enzymes is the ability to increase the rate chemical reactions occurring in living organisms, a property known as catalytic activity. Enzymes speed up the rate of reactions because they lower energy required to get to the transition state of the reaction. The transition state of the reaction is an unstable intermediate structure formed during the reaction process. For example, in Figure 7.9 showing the reaction mechanism of an ester formation, step 3 which contains 5 bonds to the central carbon atom represents the unstable transition state of this reaction. The transition state has the highest energy of the reaction and is noted on the Gibbs Free Energy Diagram as the pinnacle of the ‘hill’ that occurs between the reactant and product energies (Figure 7.15). When enzymes or catalysts are present, the transition state energy is lowered which in turn has an exponential effect on the reaction rate (Figure 7.15). Thus, enzymes can increase the reaction rate by many orders of magnitude.
Figure 7.15 Gibbs Free Energy Diagram of an Enzyme Mediated Reaction. The reaction energy of an uncatalyzed reaction is shown in red. Note that the transition state of the reaction is the most unstable part of the reaction and thus, is the position on the graph with the highest free energy. The difference in energy between the transition state and the reactants is called the Gibbs free energy of activation, commonly known as activation energy ( Δ G ‡ ). In the presence of an enzyme (blue line) The activation energy is lowered which causes an exponential increase in the reaction rate. Note that the presence of the enzyme does not change the Gibbs Free Energy of the reactants or of the products. Thus, the presence or absence of an enzyme DOES NOT determine the spontaneity of a reaction.
Figure is adapted from Fvasconcellos
Because most enzymes are proteins, their activity is affected by factors that disrupt protein structure, as well as by factors that affect catalysts in general. Factors that disrupt or denature protein structure include temperature and pH; factors that affect catalysts in general include reactant (substrate) concentration and enzyme concentration. The activity of an enzyme can be measured by monitoring either the rate at which a substrate disappears or the rate at which a product forms.
Concentration of Substrate
In the presence of a given amount of enzyme, the rate of an enzymatic reaction increases as the substrate concentration increases until a limiting rate is reached, after which further increase in the substrate concentration produces no significant change in the reaction rate (part (a) of Figure 7.16. At this point, so much substrate is present that essentially all of the enzyme active sites have substrate bound to them. In other words, the enzyme molecules are saturated with substrate. The excess substrate molecules cannot react until the substrate already bound to the enzymes has reacted and been released (or been released without reacting).
Figure 7.16 Concentration versus Reaction Rate (a) This graph shows the effect of substrate concentration on the rate of a reaction that is catalyzed by a fixed amount of enzyme. (b) This graph shows the effect of enzyme concentration on the reaction rate in biological systems at a constant level of substrate. Note that in biological systems that the enzyme concentration is much smaller than the amount of substrate present. Thus, enzyme concentration increases will never reach the saturation point in biological systems.
Let’s consider an analogy. Ten taxis (enzyme molecules) are waiting at a taxi stand to take people (substrate) on a 10-minute trip to a concert hall, one passenger at a time. If only 5 people are present at the stand, the rate of their arrival at the concert hall is 5 people in 10 minutes. If the number of people at the stand is increased to 10, the rate increases to 10 arrivals in 10 minutes. With 20 people at the stand, the rate would still be 10 arrivals in 10 minutes. The taxis have been “saturated.” If the taxis could carry 2 or 3 passengers each, the same principle would apply. The rate would simply be higher (20 or 30 people in 10 minutes) before it leveled off.
Concentration of Enzyme
When the concentration of the enzyme is significantly lower than the concentration of the substrate (as occurs in biological systems), the rate of an enzyme-catalyzed reaction is directly dependent on the enzyme concentration [part (b) of Figure 7.16]. This is true for any catalyst; the reaction rate increases as the concentration of the catalyst is increased.
Temperature
A general rule of thumb for most chemical reactions is that a temperature rise of 10°C approximately doubles the reaction rate. To some extent, this rule holds for all enzymatic reactions. After a certain point, however, an increase in temperature causes a decrease in the reaction rate, due to denaturation of the protein structure and disruption of the active site [part (a) of Figure 7.17]. For many human proteins, denaturation occurs between 45°C and 55°C. Note that human body maintains a constant temperature of 37oC. Thus, most proteins have evolved to have maximal activity around this temperature. At high temperatures, the enzymes will melt and denature causing a loss of function, whereas at lower temperatures, the protein cannot kinetically move as fast to mediate the reaction. Other species, such as those found at deep sea thermal vents, will have enzymes specialized for those environments and have different optimal temperature ranges.
Figure 7.17 Temperature and pH versus Reaction Rate (a) This graph depicts the effect of temperature on the rate of a reaction that is catalyzed by a fixed amount of enzyme. (b) This graph depicts the effect of pH on the rate of a reaction that is catalyzed by a fixed amount of enzyme.
At 0°C and 100°C, the rate of enzyme-catalyzed reactions is nearly zero. This fact has several practical applications. We sterilize objects by placing them in boiling water, which denatures the enzymes of any bacteria that may be in or on them. We preserve our food by refrigerating or freezing it, which slows enzyme activity. When animals go into hibernation in winter, their body temperature drops, decreasing the rates of their metabolic processes to levels that can be maintained by the amount of energy stored in the fat reserves in the animals’ tissues.
Hydrogen Ion Concentration (pH)
Because most enzymes are proteins, they are sensitive to changes in the hydrogen ion (H+) concentration or pH. Enzymes may be denatured by extreme levels of hydrogen ions (whether high or low); any change in pH, even a small one, alters the degree of ionization of an enzyme’s acidic and basic side groups and the substrate components as well. Ionizable side groups located in the active site must have a certain charge for the enzyme to bind its substrate. Neutralization of even one of these charges alters an enzyme’s catalytic activity.
An enzyme exhibits maximum activity over the narrow pH range in which a molecule exists in its properly charged form. The median value of this pH range is called the optimum pH of the enzyme [part (b) of Figure 7. 17]. With the notable exception of gastric juice (the fluids secreted in the stomach), most body fluids have pH values between 6 and 8. Not surprisingly, most enzymes exhibit optimal activity in this pH range. However, a few enzymes have optimum pH values outside this range. For example, the optimum pH for pepsin, an enzyme that is active in the stomach, is 2.0.
7.6 Introduction to Pharmacology
Pharmacology is the branch of medicine concerned with the uses, modes and mechanisms of action of drug molecules. The term mechanism of action (MOA) refers to the specific biochemical interaction through which a drug substance produces its pharmacological effect. A mechanism of action usually includes mention of the specific molecular targets to which the drug binds, such as an enzyme or receptor. Receptor sites have specific affinities for drugs based on the chemical structure of the drug, as well as the specific action that occurs there. Drugs that do not bind to receptors produce their corresponding therapeutic effect by simply interacting with chemical or physical properties in the body. Common examples of drugs that work in this way are antacids and laxatives. In comparison, a mode of action (MoA) describes functional or anatomical changes, at the cellular level, resulting from the exposure of a living organism to a substance.
This section will focus primarily on common drug MOAs. Drugs can act on molecular targets from any of the major macromolecule groups or from mixtures of the different groups. DNA and RNA often form complexes with proteins and many cellular receptors are modified with carbohydrate structures forming both glycoproteins and glycolipids. Drugs can have effects through the binding of molecular targets are very specified locations in the cell as depicted in Figure 7.18
Figure 7.18 Cellular Drug Targets. Drug molecules can bind many different types of cellular targets to mediate their effects. Several are indicated in the diagram above.
The figure is adapted from: The National Science Foundation
Drugs mediate their effects by acting either as agonists or antagonists. Agonists occupy the normal substrate/ligand binding site and produce a conformational change which leads to enzyme/receptor activation. Antagonists, on the other hand, occupy the active site of a receptor or enzyme, but produce no conformational change and prevent the action of the normal substrate/ligand. When studying these types of molecules, researchers are concerned with two primary properties of the drug molecule, the affinity and the efficacy of the drug. Affinity refers to how well the compound binds to a receptor, where drugs with higher affinity tend to exert greater or more long lasting effects. Efficacy, on the other hand, is a measure of how well the compound activates the receptor. Typically, strong agonists will also have high efficacy, whereas strong antagonists will have low efficacy and inhibit cellular pathways.
Antagonists
Antagonists can be further divided into subcategories based on their mechanism of inhibition. These include competitive, noncompetitive, and irreversible inhibitors (Figure 7.19) Competitive inhibitors will bind reversibly to the same binding sight as the normal ligand/substrate. They will move in and out of the active site competing with the binding of the normal substrate and thereby reducing the overall activity of the receptor/enzyme. Since competitive inhibitors don’t alter the shape of the drug target and don’t permanently block the active site of the drug target, the inhibition that they cause can be overcome by adding additional substrate.
A noncompetitive inhibitor will bind reversibly to a drug target at a distant site from the active site and cause a conformational change that will prevent the binding or activation of the target by the normal substrate. Since noncompetitive inhibitors cause conformational changes to the drug target, the inhibition that they cause cannot be overcome by adding more of the normal substrate. However, the drug target will not be permanently altered. Once the inhibitor is metabolized, the drug target will regain its proper shape and will retain its activity. This type of binding and conformational change is known as allosteric binding. An allosteric binding site is any binding site away from the active site of the drug target.
Finally, irreversible inhibitors will covalently bind to the drug target and permanently alter either the active site directly, or the conformational shape of the drug target such that it is no longer functional.
Figure 7.19 Mechanisms of Drug Target Inhibition. In competitive inhibition, the antagonist binds to the active site of the drug target and reversibly inhibits the binding of the normal substrate. In noncompetitive inhibition, the antagonist binds at an allosteric site on the drug target where it causes a conformational change in the shape of the drug target and prevents the normal substrate either from binding to the drug target (as shown above) or it effects the efficacy of the normal substrate by decreasing the drug target activation by the normal substrate. Noncompetitive inhibitors bind reversibly to the drug target and do not permanently alter the drug target. Irreversible inhibitors bind covalently to the drug target and permanently alter its activity.
This figure was adapted from BioNinja
7.7 Chapter Summary
Metabolism is the set of life-sustaining chemical reactions in organisms. Metabolic reactions may be categorized as catabolic – the breaking down of compounds, or anabolic – the building up (synthesis) of compounds. Most metabolic reactions in the body require the activity of an enzyme catalyst. The enzyme shape is critical to function. Enyzmes bind their substrates via the Lock and Key Model or the Induced Fit Model.
Common types of enzymatic reactions that occur in the body, include: Group Transfer Reactions that are mediated by Transferase Enzymes, The formation or removal of Carbon-Carbon Double Bonds by Lyase Enzymes, Isomerization Reactions mediated by Isomerase Enzymes, Ligation Reactions that combine two substrates together and are mediated by Ligase Enzymes, Hydrolysis reactions that involve the insertion or removal of water from the substrates and are mediated by a Hydrolase Enzyme, and Oxidation and Reduction Reactions that involve the movement of electrons from one compound to another by oxidoreductases.
Dehydration synthesis (mediated by hydrolase enzymes) is used to make all of the major macromolecules in the body. Lipids are formed by combining glycerol and fatty acids together with ester bonds (carboxylic acid + alcohol = ester). Carbohydrates are formed by combining sugar monomers together with glycosidic bonds (hemiacetal + alcohol = acetal). Proteins are formed by combining amino acids together in peptide bonds (carboxylic acid + amine = amide). Nucleic Acids (DNA/RNA) are formed by linking nucleotides together in phosphodiester bonds (phosphoric acid + alcohol à phosphoester).
Organic oxidation and reduction reactions commonly involve oxygen containing compounds. With regards to oxidation, Primary Alcohols can be oxidized to Aldehydes which can be further oxidized to Carboxylic Acids. Secondary Alcohols can be oxidized to Ketones. Tertiary Alcohols and Ketones CANNOT be oxidized. With regards to reduction, Aldehydes can be reduced to Primary Alcohols. Ketones can be reduced to Secondary Alcohols.
ATP is the major energy currency within the cell. It is present within the human body at very low concentrations (~ 250 g) However, the average human will use their weight (50 – 75 kg) in ATP every single day! Thus, ATP must continually be recycled within the human body alternating between ADP and ATP. ATP is recycled in the mitochondria in a process called oxidative phosphorylation. In this process, energy is taken from food molecules through oxidation. The electrons that are pulled from the food molecules can be used as an energy source to make ATP by phosphorylating ADP. Specifically, the electrons from food are used in the electron transport chain to create a proton (H+) gradient in the intermembrane space of the mitochondria. The proton gradient, flows like a river through the ATP synthase protein channel and drives the production of ATP.
Chemical bonds between atoms store energy known as bond energy. Each molecule has its own characteristic bond energy. Thus, when breaking a chemical bond, energy needs to be added to overcome the bond energy between the two atoms. When new bonds form they release energy.
For a chemical reaction to be spontaneous, the reaction needs to be exergonic, or releasing energy as a product of the reaction . If a reaction requires energy input, it is said to be endergonic and will NOT occur spontaneously. Spontaneity can be measured using the Change in Gibbs Free Energy (ΔG). This can also be represented graphically. If ΔG is negative, energy will be released and the reaction is spontaneous, whereas if ΔG is positive, the reaction requires energy input and is NOT spontaneous. If ΔG = 0, the reaction is at equilibrium and there is no net movement in either direction.
Many factors will effect the rate of a chemical reaction that is mediated by an enzyme catalyst. These include:
- The concentration of substrate – increasing substrate will increase reaction rates until all the enzyme is saturated with substrate.
- Increasing enzyme concentration will increase the reaction rate
- Altering temperature or pH will alter the reaction rates of an enzyme catalyzed reaction. Enzyme folding and movement is effected by both of these parameters. Thus, enzymes have optimal pH and temperature ranges.
Pharmacology studies the modes and mechanisms of action of drug molecules. Drug molecules can act via several different mechanism within the cell, known as its mechanism of action (MOA). Drugs can mediate their effects by acting as agonists (which mimic the normal reaction mechanisms of the body) or antagonists (which inhibit normal reaction mechanisms of the body). Drug affinity refers to how well a drug will bind its target molecule, whereas efficacy is a measure of how well the drug activates its target.
Antagonists inhibit reactions using three main mechanisms.
- Competitive Inhibition
- Noncompetitive Inhibition
- Irreversible Inhibition
In Competitive inhibition, the inhibitor binds reversibly with the active site of the enzyme and can be overcome by adding more substrate. Noncompetitive inhibitors, on the other hand, bind to an allosteric site of the enzyme, away from the active site. Therefore, they cannot be overcome by adding more substrate. Both of these mechanisms are reversible inhibitory processes. Irreversible inhibitors bind covalently to the enzyme and permanently alter the shape and function.
7.8 References
- Farmer, S., Reusch, W., Alexander, E., and Rahim, A. (2016) Organic Chemistry. Libretexts. Available at: https://chem.libretexts.org/Core/Organic_Chemistry
- Ball, et al. (2016) MAP: The Basics of GOB Chemistry. Libretexts. Available at: https://chem.libretexts.org/Textbook_Maps/Introductory_Chemistry_Textbook_Maps/Map%3A_The_Basics_of_GOB_Chemistry_(Ball_et_al.)/14%3A_Organic_Compounds_of_Oxygen/14.10%3A_Properties_of_Aldehydes_and_Ketones
- McMurray (2017) MAP: Organic Chemistry. Libretexts. Available at: https://chem.libretexts.org/Textbook_Maps/Organic_Chemistry_Textbook_Maps/Map%3A_Organic_Chemistry_(McMurry)
- Soderburg (2015) Map: Organic Chemistry with a Biological Emphasis. Libretexts. Available at: https://chem.libretexts.org/Textbook_Maps/Organic_Chemistry_Textbook_Maps/Map%3A_Organic_Chemistry_With_a_Biological_Emphasis_(Soderberg)
- Ophardt, C. (2013) Biological Chemistry. Libretexts. Available at: https://chem.libretexts.org/Core/Biological_Chemistry/Proteins/Case_Studies%3A_Proteins/Permanent_Hair_Wave
- Soderberg, T. (2016) Organic Chemistry with a Biological Emphasis. Libretexts. Available at: https://chem.libretexts.org/Textbook_Maps/Organic_Chemistry_Textbook_Maps/Map%3A_Organic_Chemistry_with_a_Biological_Emphasis_(Soderberg)
- Ball, et al. (2016) MAP: The Basics of General, Organic, and Biological Chemistry. Libretexts. Available at: https://chem.libretexts.org/Textbook_Maps/Introductory_Chemistry_Textbook_Maps/Map%3A_The_Basics_of_GOB_Chemistry_(Ball_et_al.)
- Clark, J. (2017) Organic Chemistry. Libretexts. Available at: https://chem.libretexts.org/Core/Organic_Chemistry/Amides/Reactivity_of_Amides/Polyamides
- Wikipedia contributors. (2018, December 28). Metabolism. In Wikipedia, The Free Encyclopedia. Retrieved 19:28, December 29, 2018, from https://en.wikipedia.org/w/index.php?title=Metabolism&oldid=875751739
- Ball, Hill, and Scott. (2012) Enzyme Activity, section 18.7 from the book Introduction to Chemistry: General, Organic and Biological (v1.0) retrieved on Dec 31, 2018 from https://2012books.lardbucket.org/books/introduction-to-chemistry-general-organic-and-biological/s21-07-enzyme-activity.html
- Wikipedia contributors. (2018, November 29). Mechanism of action. In Wikipedia, The Free Encyclopedia. Retrieved 05:00, January 1, 2019, from https://en.wikipedia.org/w/index.php?title=Mechanism_of_action&oldid=871201209